Luận án Synthesis and properties of undoped and transition metal (Mn₂⁺, Cr³⁺) doped Zn₂SiO⁴ and Zn₂SnO⁴ phosphors
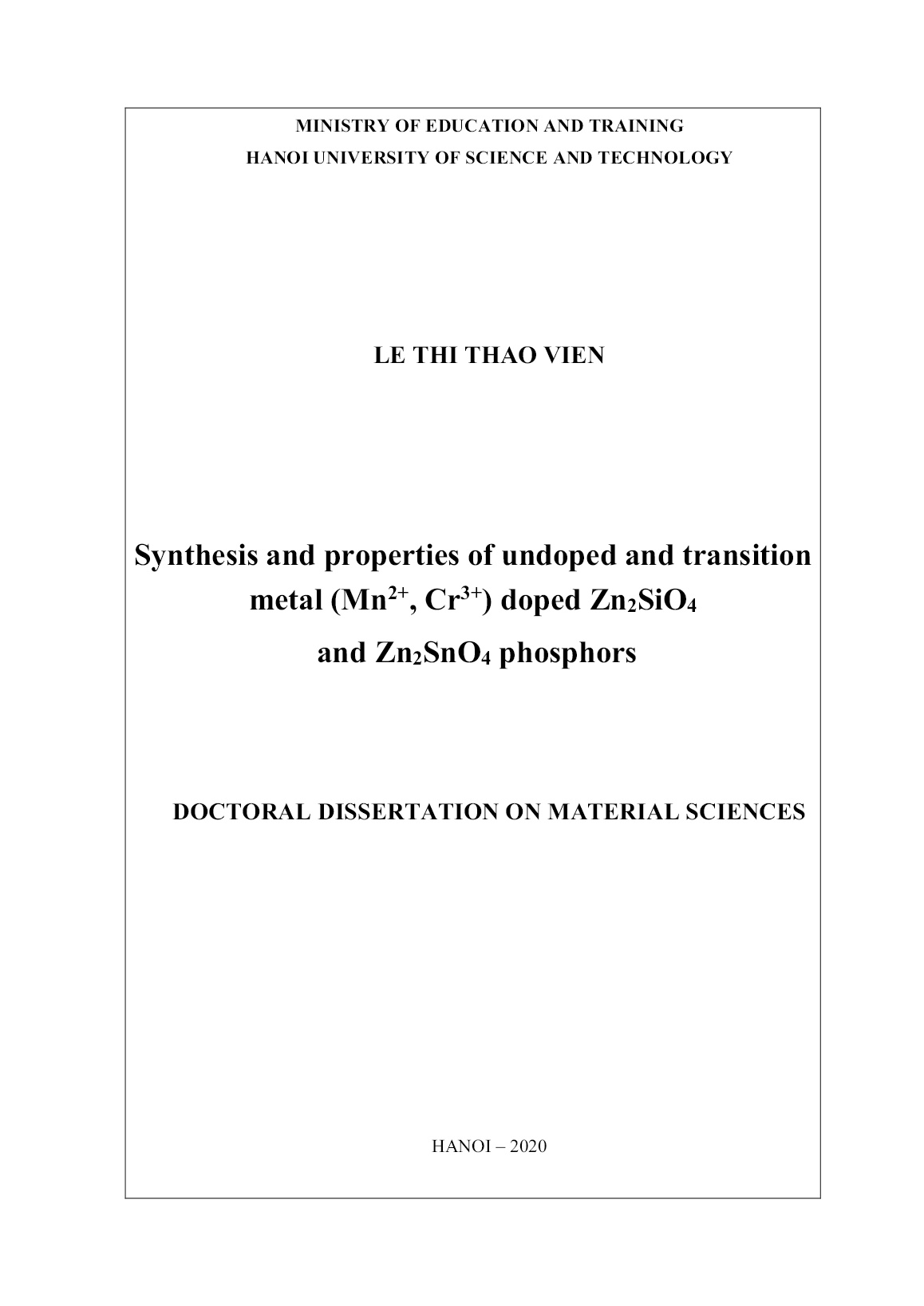
Trang 1
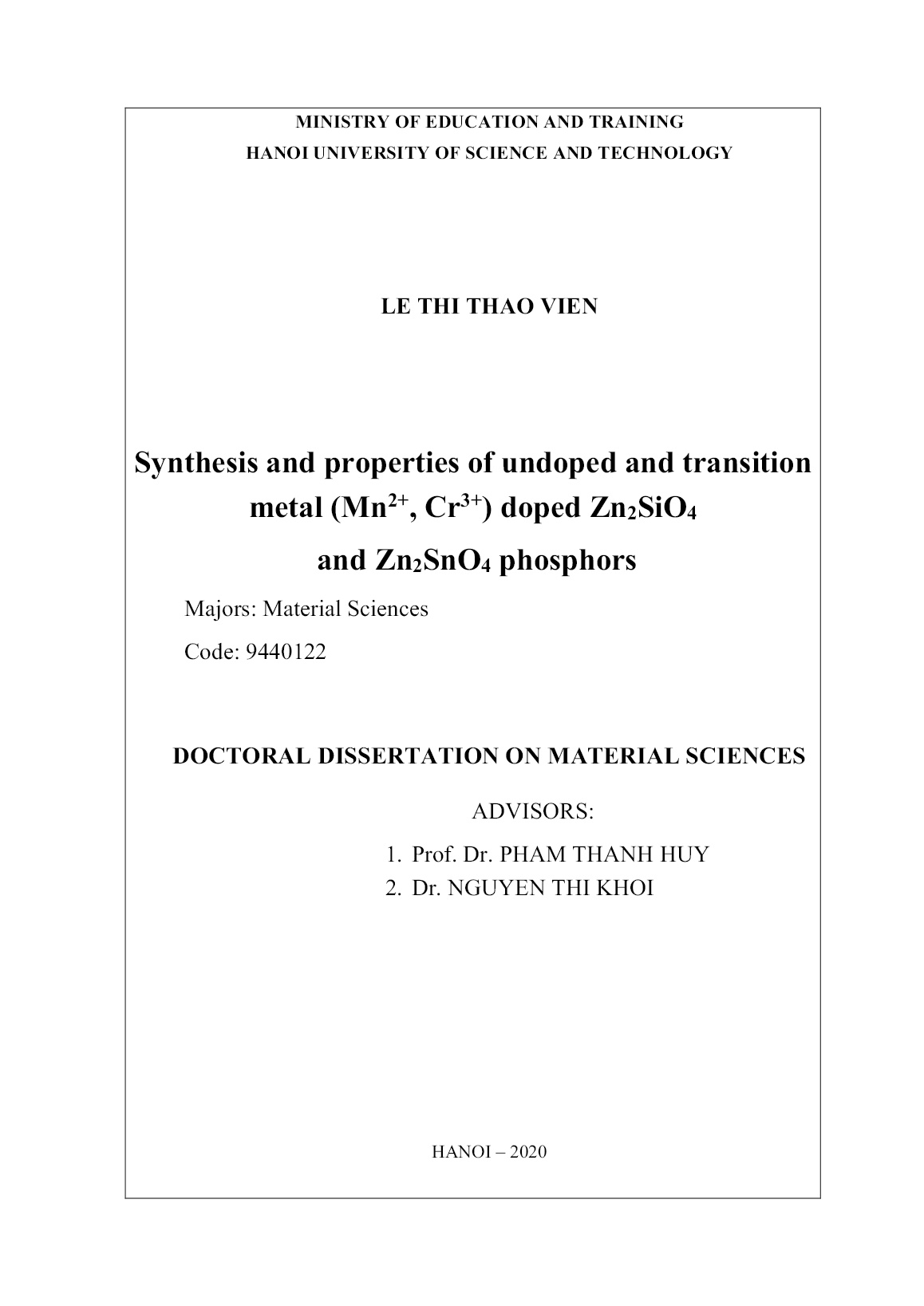
Trang 2
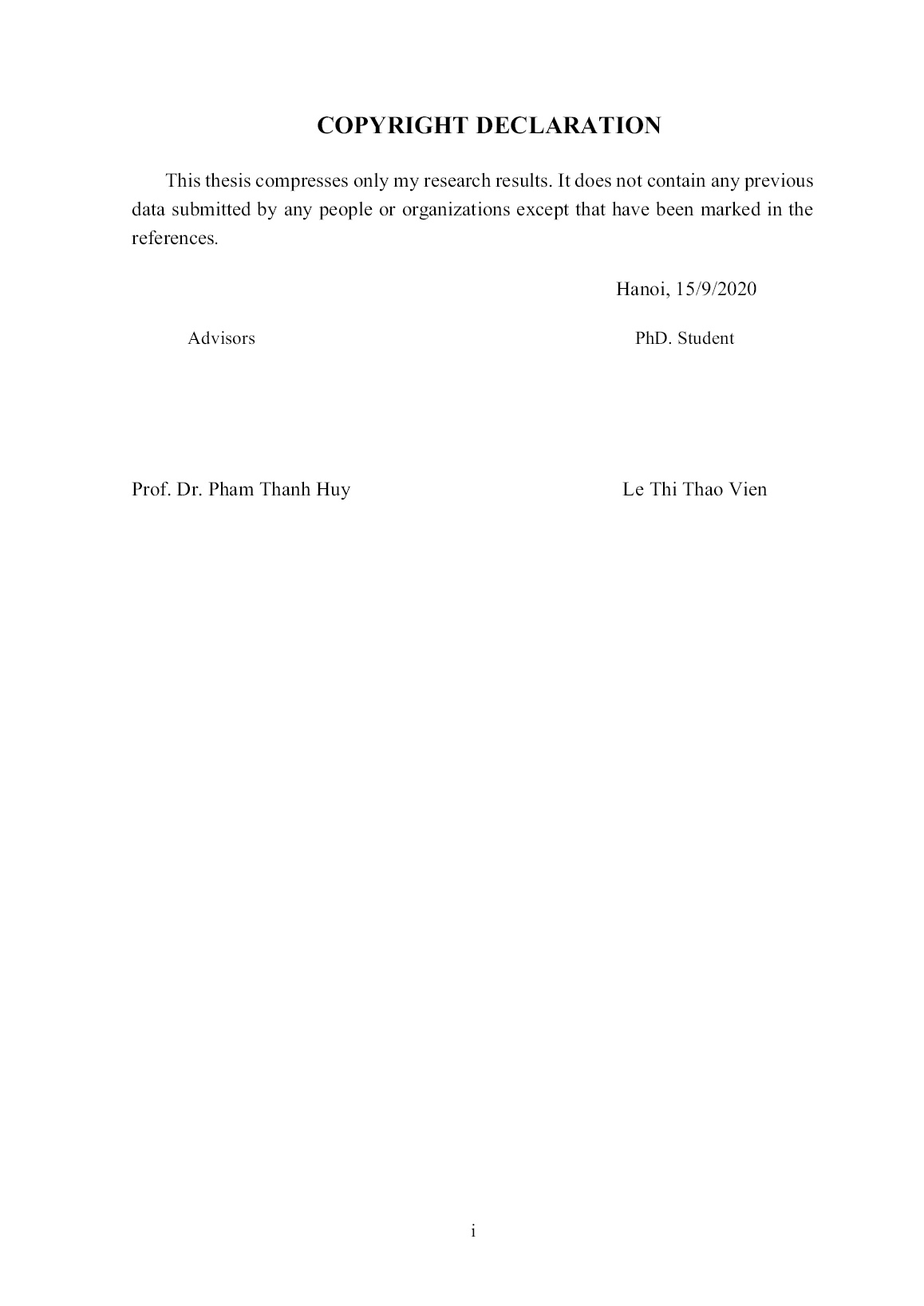
Trang 3
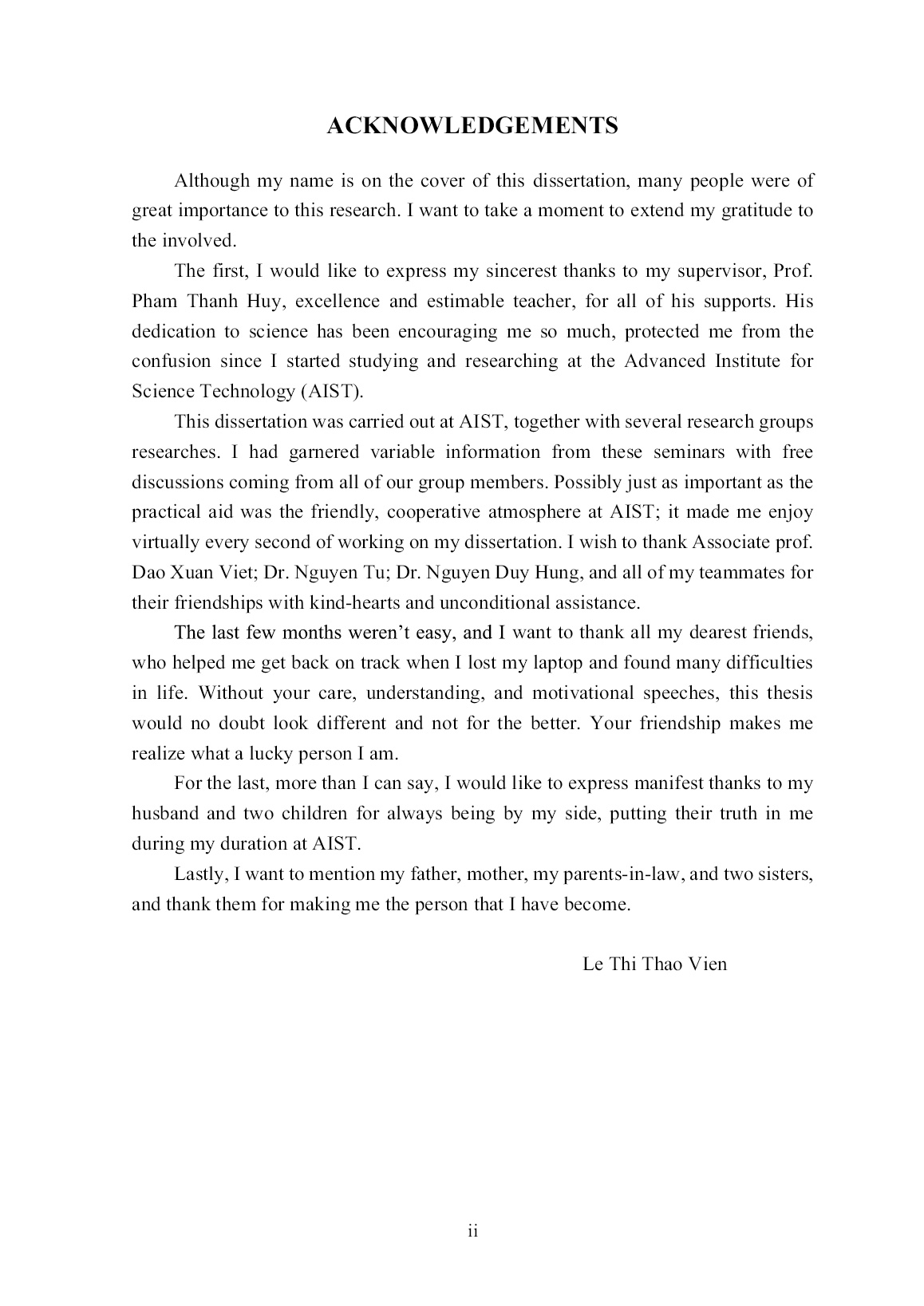
Trang 4
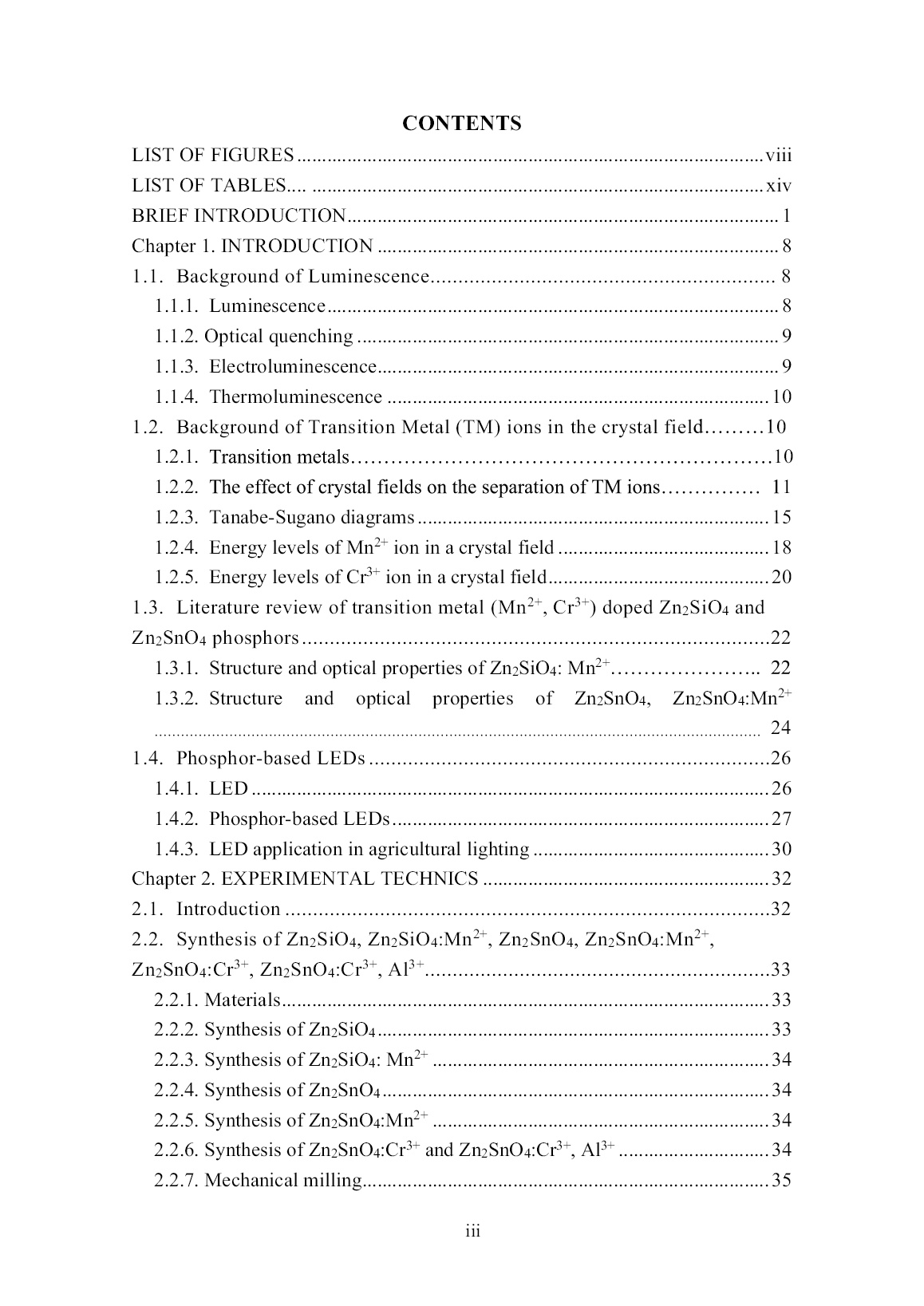
Trang 5
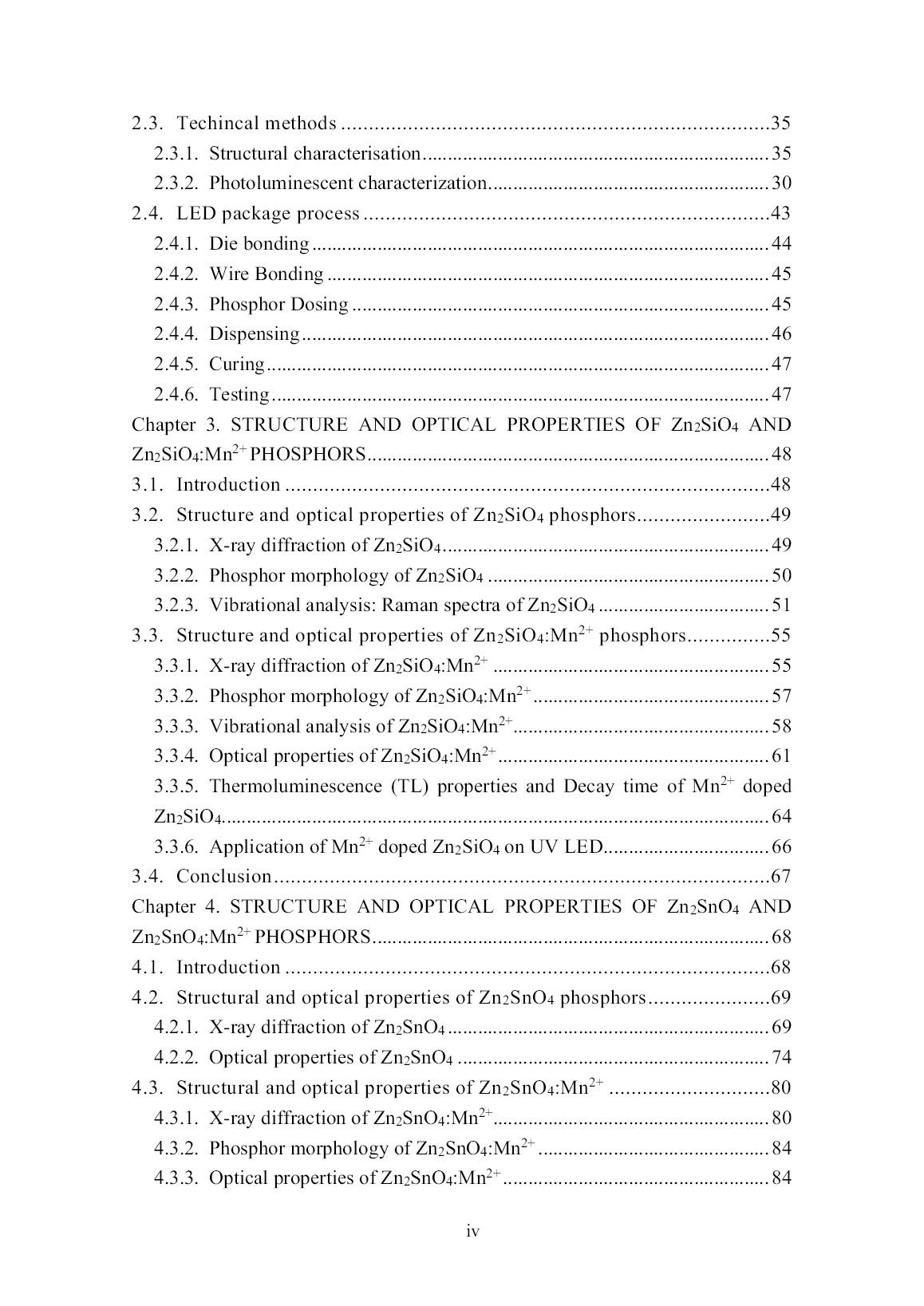
Trang 6
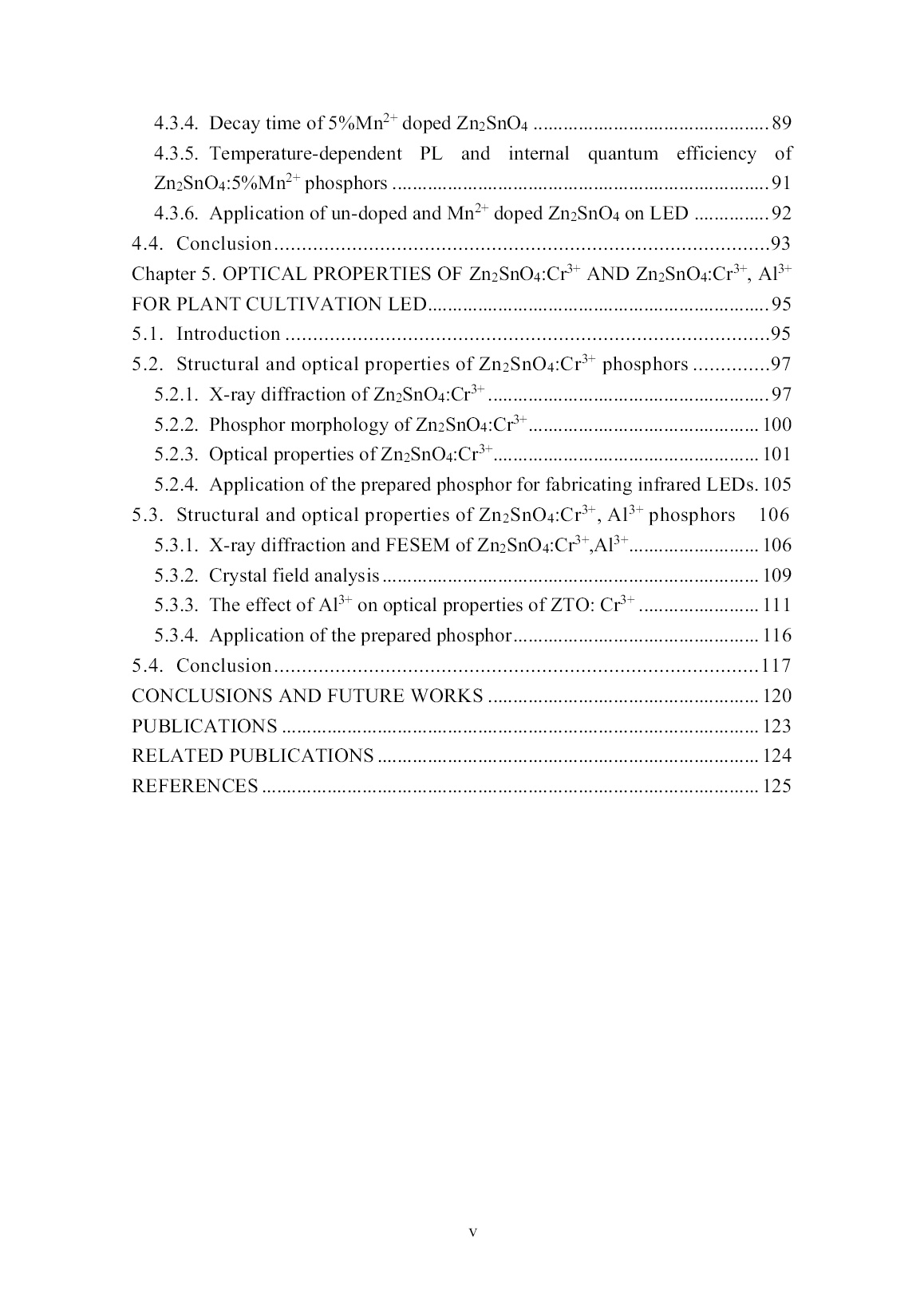
Trang 7
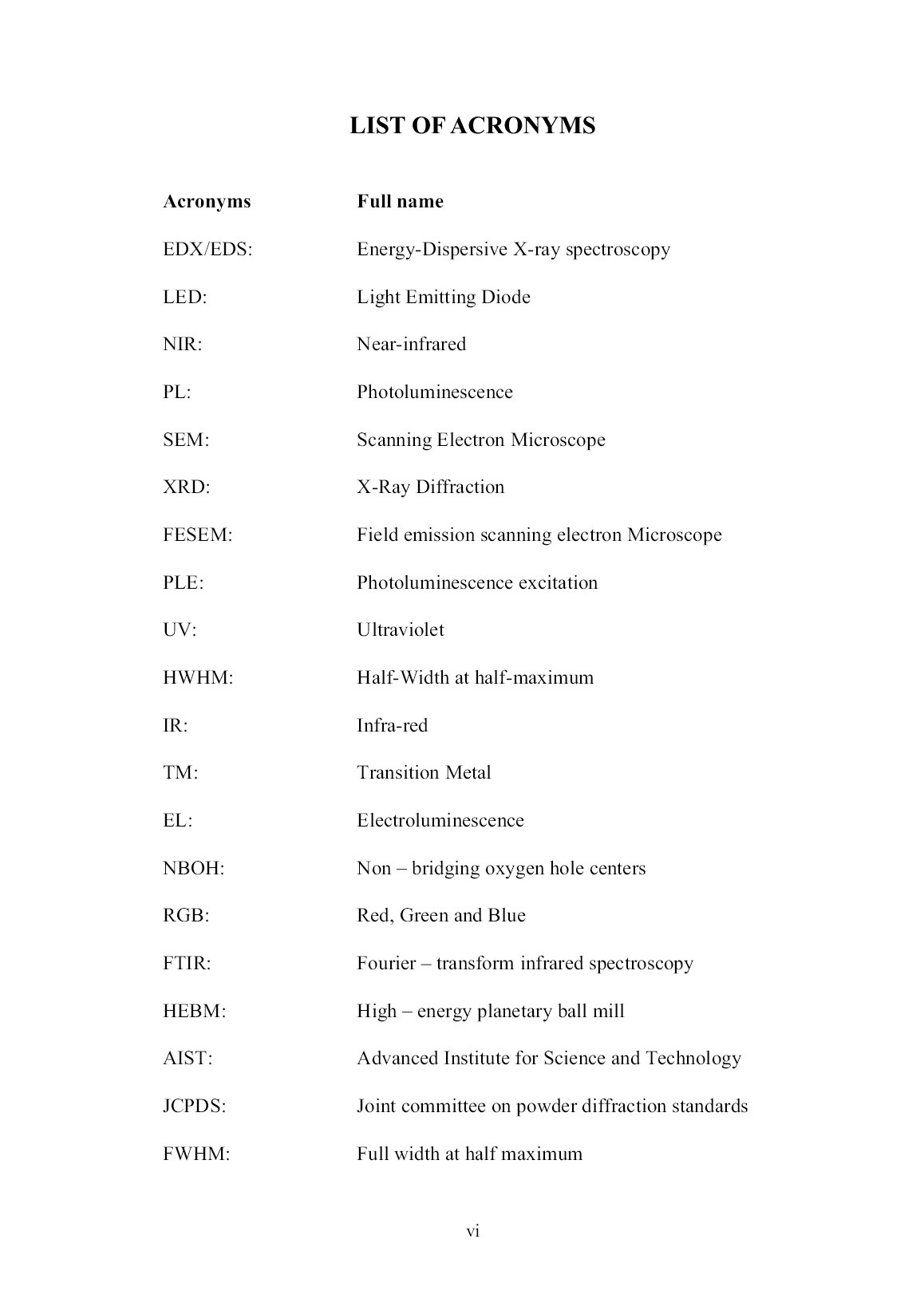
Trang 8
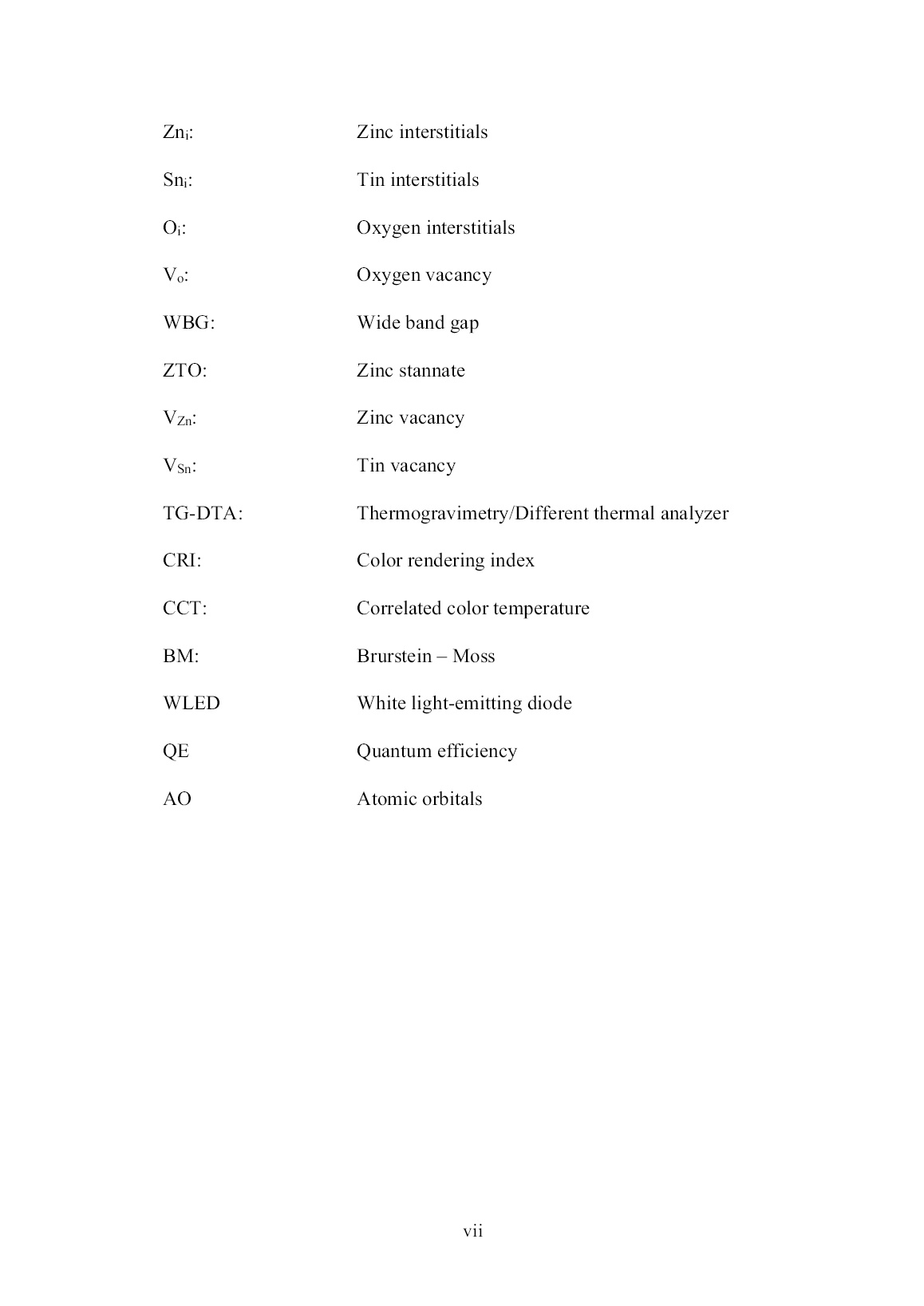
Trang 9
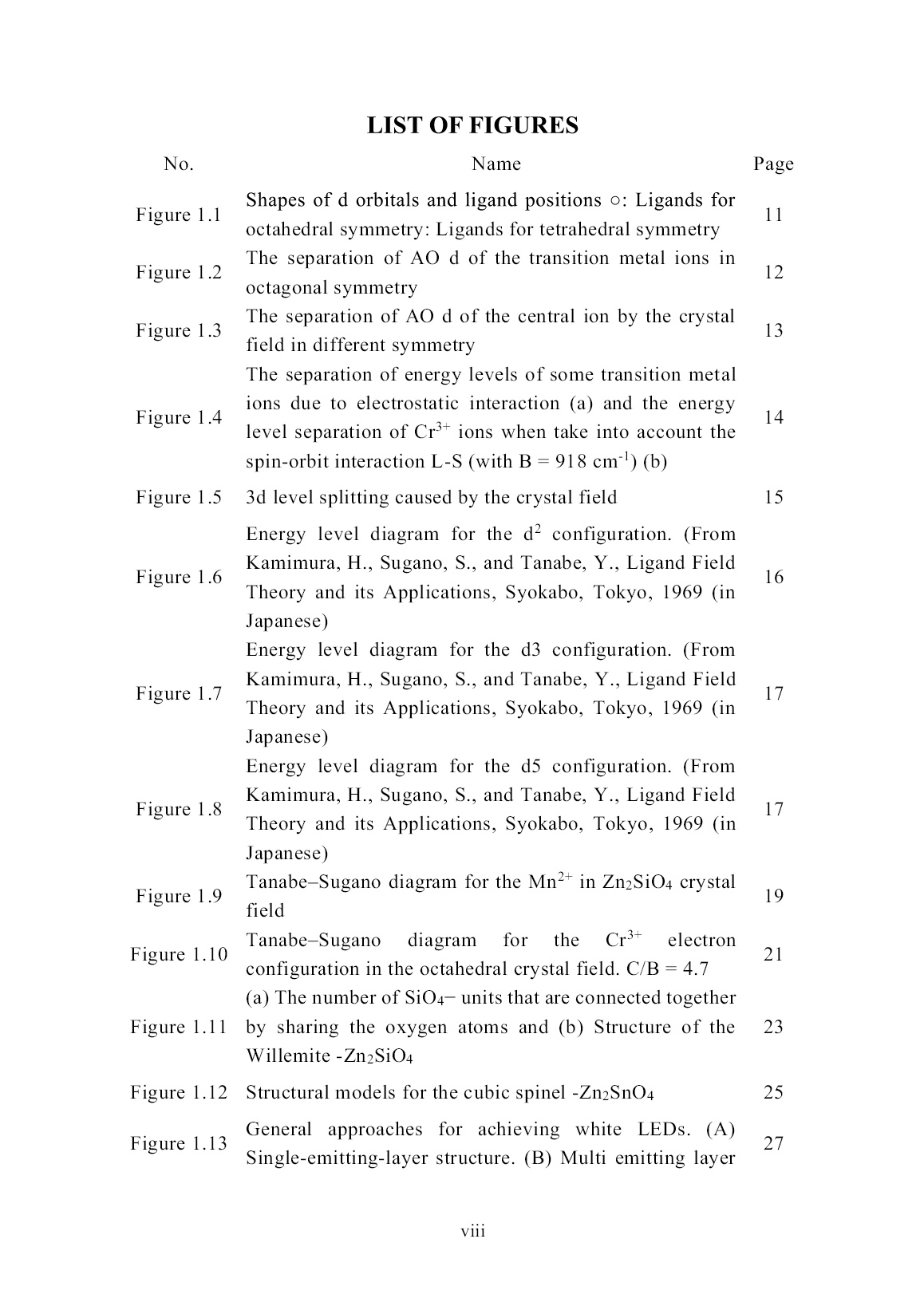
Trang 10
Tải về để xem bản đầy đủ
Bạn đang xem 10 trang mẫu của tài liệu "Luận án Synthesis and properties of undoped and transition metal (Mn₂⁺, Cr³⁺) doped Zn₂SiO⁴ and Zn₂SnO⁴ phosphors", để tải tài liệu gốc về máy hãy click vào nút Download ở trên.
Tóm tắt nội dung tài liệu: Luận án Synthesis and properties of undoped and transition metal (Mn₂⁺, Cr³⁺) doped Zn₂SiO⁴ and Zn₂SnO⁴ phosphors
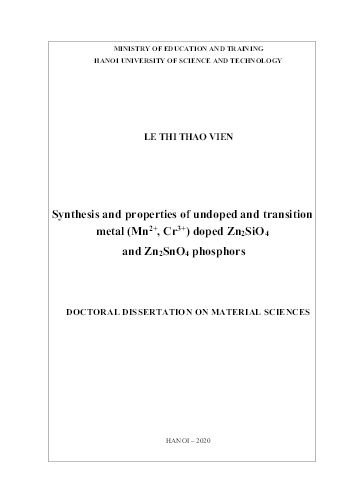
results, as has been described above. It is noticed that the vibration modes of Zn-O and Si-O-Si bonds are disappeared completely, while the stretching vibrations of the ZnO4, and SiO4 groups become sharp and well- defined peaks in the FTIR spectrum of the samples annealed above 1150 °C, as shown in Fig. 3.10(d-g). 3.3.3.2. Raman spectra Figure 3.11. Raman spectra of 5 wt% Mn2+-doped ZnO2SiO4 powders after milling for 40 hours (a), the samples milled for 40 hours followed by annealing at different temperatures of 500 °C (b), 900 °C (c), 1150 °C (d), 1200 °C (e), 1250 °C (f) , 1300 °C (g), for 2 h in air Fig. 3.11 displays Raman spectra of Zn2SiO4:5%Mn2+ powders after ball- milling for 40 hours and annealed at different temperatures for 2 hours in the air. The results show that the Raman spectra of the as-milled sample and the sample annealed at 500 C consist of several vibration modes at 320, 428, 460, 543, and 642 cm-1 which originated from ZnO and SiO2 materials (Fig. 3.11a-b)[123,132]. After annealing at 900 °C, a new and weak peak emerges in the Raman spectrum at 865 cm-1, besides the characteristic peaks of the ZnO and SiO2 (Fig. 3.11c). This peak is contributed to the surface vibrational mode of the siloxane group (the Si–O–Si linkage) in -Zn2SiO4 structure [120,131]. This result demonstrates that the - 61 Zn2SiO4 phase was formed at the annealing temperature as low as 900 °C. With further increasing annealing temperature, three peaks related to the surface siloxance group at 870, 907, 947 cm-1 appear and dominate in the Raman spectra [16,75,120,131]. The intensity of these peaks first increases with increasing annealing temperature up to 1250 C and then reduce at high temperature. This result is in good agreement with both XRD and FTIR results, as shown in Fig. 3.7, and Fig. 3.10 and revealing the optimum heat-treatment condition for the -Zn2SiO4 phase is 1250 C. 3.3.4. Optical properties of Zn2SiO4:Mn2+ 3.3.4.1. PLE and PL spectra of the un-doped and Mn2+ doped Zn2SiO4 PLE (a) and PL (b) spectra of Zn2SiO4 powders after ball-milling (without and with Mn-doped) followed by the annealing at 1250 °C for 2 hours in air are shown in Fig. 3.12. The Figure 3.12a candidates that the samples absorb the UV region strongly around 270 nm, which related to the electronic transitions from 6A1g (6S) state in Mn2+ ions to the conduction band of Zn2SiO4 host lattice. Four weak absorption peaks at 357, 379, 421 and 433 nm are also observed in the PLE spectrum of Mn-doped sample (see inset of Fig. 3.12a) and can be attributed to the electronic transitions 6A1→4E (4D), 6A1→4T2 (4D), 6A1 →4A1(4G)/4E(4G) and 6A1g→4T2 (4G) in Mn2+ ions, respectively [16]. This is an indication that the Mn2+ ions are diffused into the Zn2SiO4 lattice. The PL spectrum of the un-doped sample consists of a strong, broad and intense near-infrared emission band centered at around 735 nm, and a weak green emission band at 520 nm (see Fig. 3.9b). The NIR peak at 735 nm is attributed to the energy transition from non-bridging oxygen hole centers (NBOHs) of SiO2 to the zinc interstitial (Zni) and oxygen vacancy (Vo) states of Zn2SiO4 [26,71,75,125]. The green peak at 520 nm is explained as due to defect-related emission in ZnO, SiO2, or/and Zn2SiO4 [133-135]. For the sample doped with five wt% Mn2+, the PL spectrum reveals an intensive green emission band peaking at around 525 nm. This emission band is the well-known green emission band of α-Zn2SiO4:Mn2+ and is originated from the electronic transitions 4T1-6A1 in Mn2+ ions, [71,75,118,125,135]. Here, the disappearance of both NIR and 520 nm emission bands upon Mn2+ doping can be explained as due to the extinction of all defects because of charge compensation or oxygen vacancies compensation. When Mn2+ ions are introduced into the host lattice, they could either substitute for Zn2+ sites in Zn2SiO4 or stay at interstitial sites that lead to the reduction of oxygen vacancies and compensate surface charge defects. Alternatively, the more efficient energy transfer process from host defects (donor) to the Mn2+ luminescence center (acceptor) that results in strong 525 nm green emission would also be the cause of the disappearance of defect-related emissions [73,79]. 62 Figure 3.12. (a) PLE spectra measured at maxima of the emission at 740 nm (curve 1) and 525 nm (curve 2); and (b) photoluminescence spectrum of Zn2SiO4 sample after milling without doping and doped with 5% Mn 3.3.4.2. Effects of dopant concentration and annealing temperature on photoluminescence of Mn2+ doped Zn2SiO4 Figure 3.13. PL spectra of 5 %wt Mn2+ doped Zn2SiO4 powder after milling for 40 hours followed by the annealing at different temperatures in the range of 500-1350 C Effects of dopant concentration and annealing temperature on the photoluminescence of Zn2SiO4: Mn2+ are also investigated, and results are shown in 735 63 Fig. 3.13, and Fig. 3.14, respectively. It is clearly seen that the PL intensity first increased with increasing annealing temperature and reached the maximum at 1250 C, and then reduced at higher annealing temperatures (Fig. 3.13). Since the luminescence intensity is dependent on the crystalline quality of the host lattice, from the XRD, FTIR, Raman as described above, we can conclude that the enhancement of the PL intensity is due to the improvement of the crystalline quality of the phosphor [45,136]. Figure 3.14 shows PL emission spectra of -Zn2SiO4 samples doped with Mn2+ ions at different concentrations. The -Zn2SiO4 sample with a Mn doping concentration of 5 wt% presented the maximum PL intensity. The reduction of the PL intensity at higher doping concentration is due to concentration quenching effect that reduces the radiative recombination process of Mn2+ ions, and therefore reduce the PL intensity [16,131]. Figure 3.14. PL spectra of Zn2SiO4:x%Mn 2+ (x=0-8) samples after milling followed by the annealing in air at 1250 C. The inset displays the dependence of the PL intensity on Mn2+ doping concentrations Besides, to demonstrate the advantage of our synthesis method, Zn2SiO4 powder doped with 5 wt% Mn2+ was also prepared by using conventional solid-state reaction method under similar experimental conditions. The PL emission spectra measured under the same conditions of the two samples prepared by the two methods are plotted in Fig. 3.15. It is shown the PL intensity of the sample produced by the modified method is nearly 1.8 times higher than that of the sample obtained by conventional solid-state reaction method. Here, the high-energy planetary ball-mill step may help 64 to improve mixing of Mn2+-doped source into the host powder before high temperature diffusion process. Figure 3.15. Comparison of the PL emission spectrum of the -Zn2SiO4:5%Mn2+ sample prepared by HEBM-SSR method with that of the sample prepared by conventional solid- state reaction method 3.3.5. Thermoluminescence (TL) properties and Decay time of Mn2+ doped Zn2SiO4 Thermoluminescence (TL) spectroscopy has emerged as an important technique that can be used to understand the dynamics of electron trapping centers. In this study, TL properties of the phosphor Zn2SiO4:5%Mn2+ phosphor was investigated by β-ray beam (Sr-90) source irradiation. Fig. 3.16a shows the TL glow curve for five wt% Mn2+-doped Zn2SiO4 with different β-ray exposure time at a constant heating rate i.e., 2 C.s-1. The sample reveals two peaks at 158 C and 235 C (see Fig. 3.16b) and linear response with a dose up to 25 minutes β-ray exposure time. The low temperature and high-intensity peak (at 158 C) can be attributed to traps of Mn2+ [64,137] while the weak and high-temperature peak (at 235 C) may be due to non- bridging oxygen (NBOHs) on which holes are trapped when the sample is β-ray irradiated. Here, similar to the PL results, no defect-related TL spectra were observed in the Mn2+-doped Zn2SiO4 sample. This is generally due to the competition between the radiative and nonradiative center or between different kinds of trapping centers [137]. As the main basis in the thermoluminescence dosimetry (TLD) is that TL output is directly proportional to the radiation dose received by the phosphor and 65 hence provides the means of estimating the dose from unknown irradiations [64]. Thus, the TL results obtained in our study show a high potential application of the Mn2+-doped Zn2SiO4 in TLD. Figure 3.16. TL spectra of Zn2SiO4:5%Mn 2+under various β-ray exposure time(a) and decomposition of glow curve into individual peaks (b) The estimated kinetic parameters (E, s, b, µg) for Zn2SiO4:5%Mn2+ phosphor is examined by using curve fitting of experimental data (see Fig.3.16b), and the results are shown in Table 3.3. As can be seen from table 3.2, the activation energy of the two peaks is 0.78 eV and 1.19 eV, and the frequency factor is in the range 1010 (s-1). These parameters give valuable information about the mechanism responsible for the emission in the present phosphor. Further, we have measured the thermoluminescence emission spectra at 158 °C and 235 °C and the result, as depicted in Fig. 3.17a confirmed that the green emission at 525 nm of the Zn2SiO4:5%Mn2+ phosphor could be observed at both temperatures. This is in good agreement with previously reported experimental results [38]. It concludes that both the traps at 0.78 eV and 1.19 eV belong to energy states in the band gap, which act as electron traps for energy transfer to Mn2+ and cause green emission. In addition, the lifetime that electrons were trapped in the traps was also measured. As shown in Fig. 3.17b, the decay time of the Mn2+ doped sample is 10.5 ms, while the un-doped Zn2SiO4 sample shows a very fast decay, which accounts for nearly 1000 ns (inset Fig.3.17b). In our opinion, the reduction of the distance of neighbouring ions because of defects in the host lattice combined with Mn2+ states in the Mn2+-doped sample may be responsible for the longer decay time. 66 Figure 3.17. Thermoluminescence emission spectra measured at 158 and 235 oC (a) and the decay curve of the Zn2SiO4:5%wt Mn 2+ phosphor (b). The decay curve of Zn2SiO4 sample is shown in the inset of Fig. 3.14b Table 3.2. Kinetic parameters of Zn2SiO4: Mn 2+(5% wt) phosphor evaluated using R. Chen method. Peaks T1(°C) Tmax(°C) T2(°C) µg b E (eV) s (s-1) Peak 1 126 158 192 0.515 general 0.78 672.109 Peak 2 212 235 283 0.693 general 1.19 1129.105 3.3.6. Application of Mn2+ doped Zn2SiO4 on UV LED Figure 3.18. Electroluminescence spectrum (a) and the CIE coordinate plot of the prototype green-emitting LED under drive current of 60 mA (b). The inset of Fig. 12b is the digital image of the actual green-emitting LED 67 In order to explore the potential application of Mn2+-doped Zn2SiO4 phosphor prepared by HEBM-SSR method, green emission LED is assembled by coating 5 wt%Mn2+-doped Zn2SiO4 phosphor on 270 nm UV-LED. The image of the green LED device underdrive current of 60 mA, and its CIE chromaticity coordination is displayed in Fig. 18. Its CIE coordinates of (0.2477; 0.6829) were located in the green wavelength range, and especially, it exhibited strong green emission under 270 nm UV excitation, which can be clearly seen with the naked eyes. 3.4. Conclusion Near-infrared Zn2SiO4 and green Zn2SiO4: Mn2+ phosphor have been produced successfully by the high-energy ball milling technique followed by annealing temperature of 1250 C in air. The PL intensity of Zn2SiO4: 5%Mn2+ produced by the modified method is nearly 1.8 times higher than that of the sample obtained by conventional solid-state reaction method. Zn2SiO4 phosphor emits a broad spectrum in the infrared (maximum at 735 nm) due to the overlap of two emission bands with the maximum of 730 and 760 nm, respectively. The origin of these two emission peaks is explained by the non-bridging oxygen defects in the Zn2SiO4 lattice. The Mn2+-doped Zn2SiO4 phosphor emits an intense green band at 525 nm. The TL glove curve of Zn2SiO4: 5%Mn2+ shows a strong peak at 158 °C and a shoulder at 235 °C, and displays linear dose-response with β-ray exposure time which indicates the phosphor could be useful for the dosimetric application. A green LED device was fabricated by using a 270 nm UV LED chip combined with 5% Mn2+-doped Zn2SiO4 phosphor, which provides 525 nm green light with CIE chromaticity coordinates of (0.2477; 0.6829) and the color purity of nearly 85%. 68 Chapter 4. STRUCTURE AND OPTICAL PROPERTIES OF Zn2SnO4 AND Zn2SnO4:Mn2+ PHOSPHORS 4.1. Introduction Light-emitting devices such as LEDs, WLED, and other optoelectronic devices based on wide band gap (WBG) semiconductor oxide materials are showing high efficiency and usefulness in recent years because of their stable physical thermal and chemical properties [110,138]. Zinc stannate (Zn2SnO4) is an essential n-type semiconductor with a band gap of about 3.67 eV [139]. In recent years, Zn2SnO4 (ZTO) has attracted great attention from many research groups because of their excellent sensing performance [2,3], superior optical, optoelectronic, and photoelectric properties [141]. Therefore, ZTO has been used in a wide range of applications such as sensors [142], batteries [143], transparent thin films [144], solar cells [145], and photocatalyst [60]. There are several methods for synthesizing ZTO, such as sol-gel [146], hydrothermal [147], sputtering [148], and solid-state interaction [149]. Among them, the solid-state interaction method shows a lot of advantages, such as mass production, less toxic, and especially high optical efficiency. In addition, there are various structural morphologies of Zn2SnO4, such as wires [150], belts [151], particles, and hybrid structures [152]. These structures are strongly dependent on experimental conditions or synthesis methods, and they are all directed towards specific applications [1,4,17]. Interestingly, different structures also have their unique optical properties and can be tunable by controlling their internal-defect states. Thanks to a large band gap (3.67 eV), a near-ultraviolet emission peak around 340 nm can be emitted by Zn2SnO4 [139]. In semiconductor materials, there are two defect types as either intrinsic or extrinsic defects due to impurities (doped elements). By optimizing the intrinsic defects of Zn2SnO4, emissions in the visible region from blue [154], green [155], yellow-orange [139], red [156] and even far-red [85] have been obtained and reported. These emissions originated from various causes such as oxygen vacancies (VO) [15,19], zinc vacancies (VZn) [1,22], tin vacancy defects (VSn) [16,18], Zn-interstitial (Zni) [20,23], Sn-interstitial (Sni) [159], lattice distortion or oxygen vacancy interactions (VO) and zinc interstitial (Zni), interstitial oxygen (Oi) in the lattice [1,20]. Red or far-red emission of Zn2SnO4, however, has been rarely observed in works of literature. Besides, the origin of these emissions has not been clearly explained yet, and they strongly depend on fabrication methods. By the Gaussian fitting method, Hsiu-Fen Lin et al. reported an emission peak at 671.1 nm from Zn2SnO4 powder synthesized by thermal plasma method, and it could be related to the Zn-interstitial (Zni) [139]. Annamalai et al. [153] and Jun Zhang et al. [160] 69 proposed that the emission peak at 680 nm from Zn2SnO4 nanoparticles fabricated by hydrothermal method and chemical vapor deposition (CVD) method,respectively are caused by zinc or tin vacancies (VZn, VSn). However, the red or far-red emission of Zn2SnO4 has not been clearly observed, and its origin is still controversial. It is interesting to notice that the high-energy planetary ball milling technique could be used to create new defects-related states [161], which could lead to new emission bands in ZTO structures. In the past years, Zn2SnO4 doped with various ions as Eu3+, Cr3+, Dy3+, Ti4+, Co2+, Ni2+, and Cu2+ have been investigated for phosphor and photocatalytic activity application [19,82,162]. Recently, Mn ions are common non-rare earth luminescence centers that have been widely used in phosphor material. Beside Mn4+ ion-doped phosphors, Mn2+ ion-doped materials have been investigated the luminescence behaviours intensively [52,163]. According to reports, the low energy levels of Mn2+ ions strongly depend on the co-valency interaction with the host crystal or the crystal field. In a suitable host lattice, Mn2+ may tuneable emission color from blue to red region due to its 4T1→6A1 transition. Thus, Mn2+ ions are an ideal non-rare earth luminescent centers for phosphor material. Until now, Mn2+ ions doped CaAlSiN3, ZnAl2O4, Zn2SiO4, Zn2GeO4, MgGa2O4, CaCl2, CdSiO3, NaCaPO4, CaZnOS had been studied as phosphors used in fluorescent lamps and WLED [164]. Although there were researches about the synthesis, investigation of magnetic and electrical properties of Zn2SnO4: Mn2+, most of the researches have not yet been reported optical properties so far. Our point, however, that the aspect is essential and deserves an optical properties investigation to extend the application of this material. In this work, we report the undoped and Mn2+ doped Zn2SnO4 synthesized by a high energy planetary ball milling technique followed by calcination in air. The crystal structure, morphologies elemental analysis, and luminescence properties of Zn2SnO4: Mn2+ phosphors were investigated in detail. 4.2. Structural and optical properties of Zn2SnO4 phosphors 4.2.1. X-ray diffraction of Zn2SnO4 4.2.1.1. Effect of annealing temperature on structural formation Figure 4.1 shows the XRD patterns of ZnO/SnO2 powder after milling for 60 hours and annealing in the range of 600-1200 °C in air. On the one hand, some featured diffraction peaks are observed at 26.57°, 33.87°, 37.94°, 51.75°, 54.75°, 61.88°, and 64.74°, as shown in Figure 4.1a. They respectively correspond to the crystal planes (110), (101), (200), (211), (220), (310), and (112), which belong to the face-centered structure of SnO2 material (JCPDS card No. 00-021-1250) [165]. On the other hand, the diffraction peaks of the wurtzite structure of ZnO are also observed 70 at 31.71° and 36.25°, corresponding to the (100) and (101) plane, respectively (JCPDS card No. 00-005-0664] [166]. At 600 ˚C, no noticeable change in the XRD peak position can be observed when compared with the as-milled sample (see Figure 4.1b), suggesting no reaction between ZnO and SnO2 occurs at this temperature. However, when the annealing temperature is increased up to 700 ˚C, three new peaks are observed in the XRD pattern (Figure 4.1c) at 34.29°, 41.68° and 60.40°, corresponding to the (311), (400) and (440) planes of the inverse spinel structure of Zn2SnO4 (JCPDS standard No. 00-024-1470). Table 4.1. The phase composition of the obtained samples annealed at different temperatures in air Sample Phase composition As-milled SnO2 ZnO - 600 °C SnO2 ZnO - 700 °C SnO2 ZnO Zn2SnO4 800 °C SnO2 ZnO Zn2SnO4 900 °C - - Zn2SnO4 1000 °C - - Zn2SnO4 1100 °C - ZnO Zn2SnO4 1200 °C - ZnO Zn2SnO4 When the samples are annealed in the range of 800-1200 °C, the intensity of diffraction peaks first increases to reach the maximum value at 1000 °C (Figure 4.1d- f) and then decreases with higher calcination temperatures. It is worthy to notice that ZnO and SnO2 phases disappear at 900 °C (Figure 4.1e), indicating a complete interaction between them. However, the ZnO phase is observed to reappear at high
File đính kèm:
luan_an_synthesis_and_properties_of_undoped_and_transition_m.pdf
thong tin mới luận án - tiếng Anh.pdf
thong tin mới luận án- Tiếng Việt.pdf
Tom tat LA Tieng Anh-Le Thi Thao Vien-2020.pdf
Tom tat LA -tiếng việt-Lê Thị Thảo Viễn.pdf